Astronomy is all about light. Of course, when we say ‘light’, we mean the complete span of the electromagnetic spectrum, of which only a tiny proportion is visible to the naked eye.
But astronomy can also be about sound. As we all know, sound waves are different to light waves.
Light waves can pass through many media, depending on their wavelength and the medium’s properties.
Light waves don’t actually require a material to travel through, and so the light from stars is unhindered by the vacuum of space.
For more on astronomical audio, read our guide on how astronomers listen to the sound of stars.
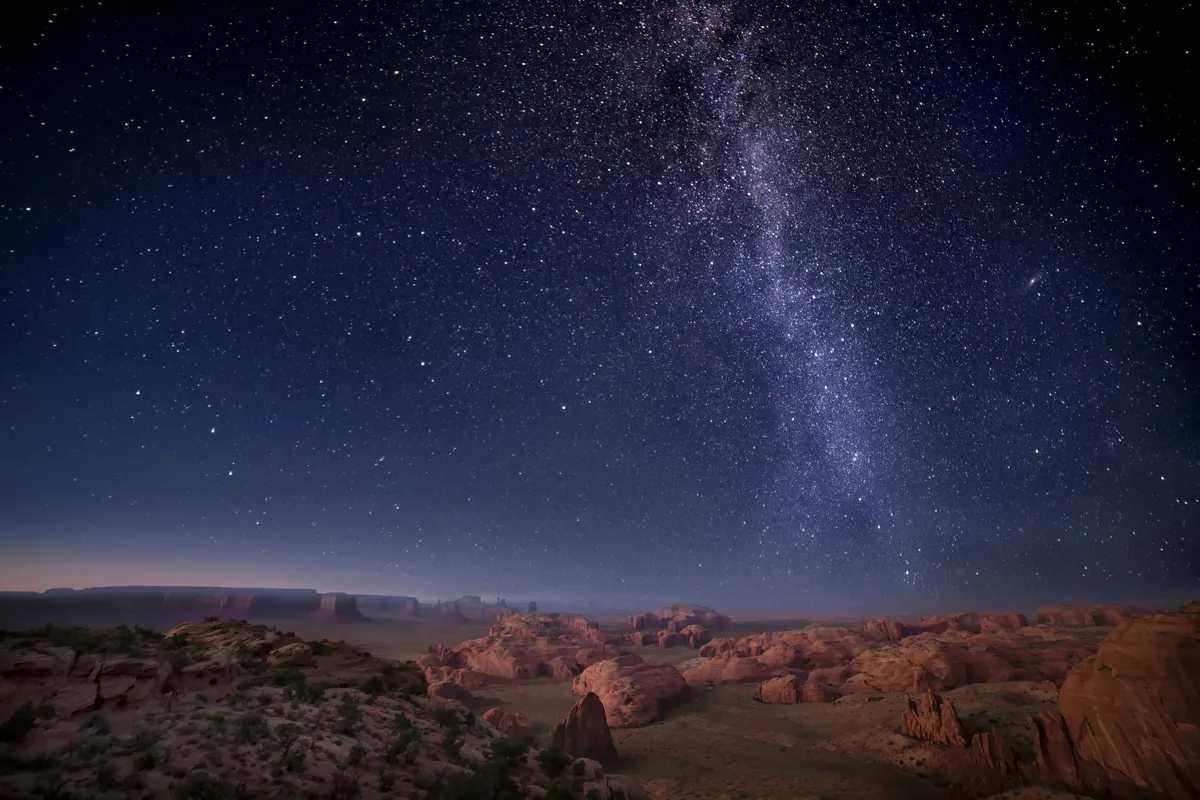
Sound waves, however, involve the compression and rarefaction of matter.
The vibration of the surface of a drum, for example, also makes the air molecules vibrate.
These in turn cause adjacent air molecules to vibrate and so a sound wave travels outward from the drum.
In a vacuum, there are no molecules in contact with the surface of a struck drum, so a microphone next to it will pick up absolutely nothing, no matter how hard the drum is banged.
So how do astronomers hear any sound at all in space?
They do it using a technique called sonification, where data is converted into sound to allow it to be interpreted.
The humble Geiger counter is an excellent example of this, measuring radiation levels and portraying the results as audio.
Just as X-ray data from space is often turned into coloured images, various astronomical data can be usefully ‘sonified’.
Can sounds travel through space?
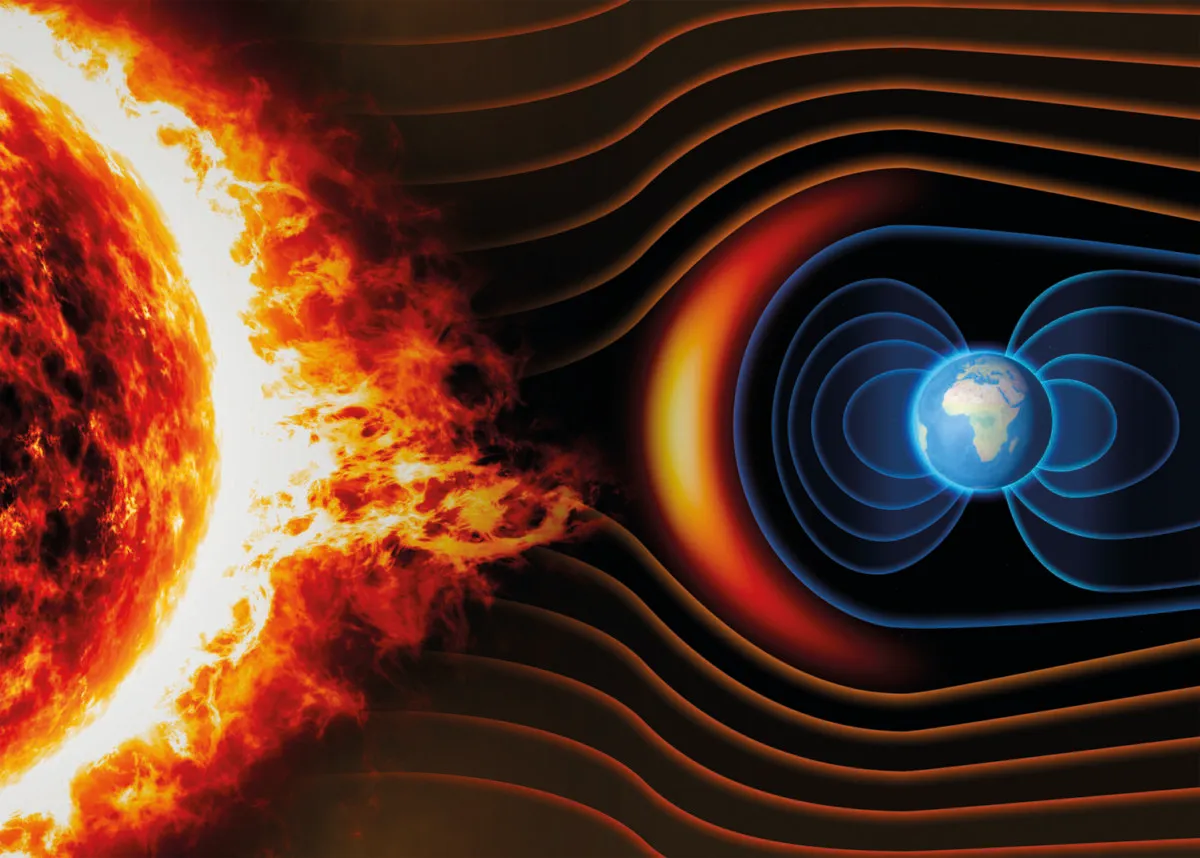
Are there actually sounds in space? If so, how can they be heard, and how do astronomers know they're there?
Space is not a perfect vacuum: the stream of charged particles flowing from the Sun – the solar wind – pervades the Solar System with an extremely thin gas in which various ethereal phenomena occur.
Unlike the gas in our atmosphere, which is bound together by the mutual collisions of air molecules, this plasma is bound together by the magnetic field surrounding it.
The turbulent nature of the Sun’s surface means that pressure and density disturbances travel outwards at the speed of sound in the solar wind.
Due to the temperature and density of the plasma, this is around a few hundred kilometres per second.
These pressure disturbances are effectively sound waves in interplanetary space.
The thin plasma of the solar wind, along with the solar magnetic field that binds it, becomes weaker and even more sparse as you travel outwards into the depths of the Solar System.
Eventually it becomes so weak that it is overwhelmed by the combined magnetic fields and particles from all the other stars in the Galaxy.
This zone, known as the ‘heliopause’, marks the end of the Sun’s dominion and is the true edge of the Solar System.
Below we've taken a look at some bodies of the Universe capable of making sound, and how astronomers are able to hear them.
Jupiter
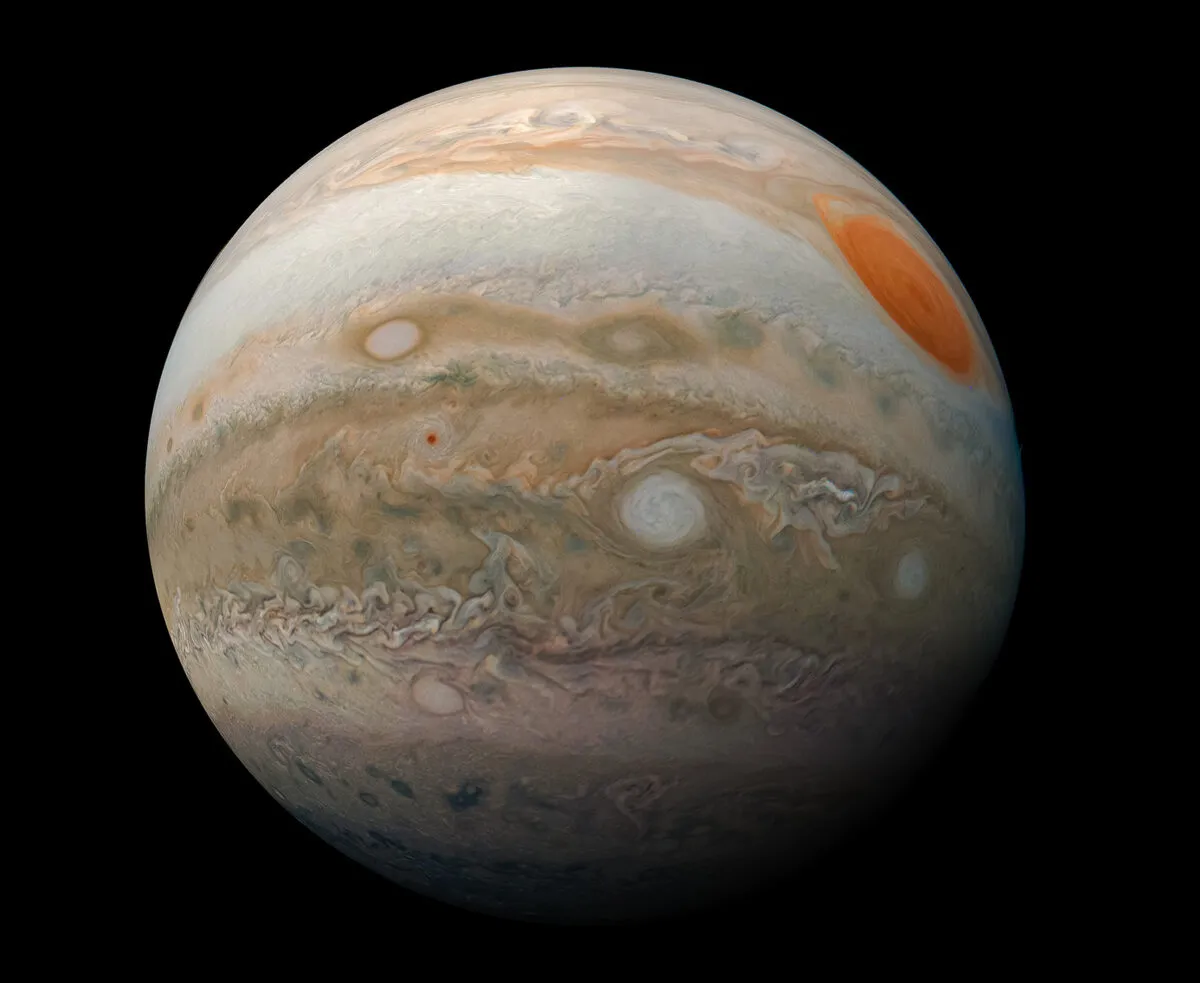
Probably the most widespread use of sonification is by amateur astronomers and radio enthusiasts, who regularly tune in to Jupiter using short-wave receivers and antennae connected to speakers.
First discovered in 1955, the radio sounds from Jupiter are diverse.
They have been described variously as sounding like waves crashing on a beach, woodpeckers and whale song.
The broadcasts from ‘Radio Jove’ come from natural radio lasers, caused by electrically conducting gas spewed into Jupiter’s magnetic field by volcanoes on its satellite Io.
This gas collects in a doughnut-shaped torus around Jupiter and, as Io ploughs through it, creates magnetic ‘Alfven’ waves.
These waves move along the lines of magnetic force in Jupiter’s field and transmit a staggering 40 trillion watts of power to its polar regions, powering the radio lasers that periodically sweep across the Earth.
The Galileo mission at Jupiter

However, it is not only amateurs who use sonification in astronomy. The epic Galileo mission, which operated for nearly 8 years in Jupiter orbit, used sonification to present some of its results.
In June 1996, Galileo passed close to the planet-sized moon Ganymede, and the onboard Plasma Wave Experiment discovered the presence of a magnetic field surrounding the moon – the first discovered in the Solar System.
The flyby lasted a couple of hours, and when the magnetic data is compressed so that 45 minutes of spacecraft time becomes a sound file just one minute long, you can hear the loud burst of noise when Galileo enters the moon’s magnetosphere.
As the spacecraft closes on Ganymede you hear a gradually rising tone that goes on to decline.
This provides a direct measure of the density of charged particles in the space around Ganymede.
The Sun

Closer to home, we can listen in to the Sun using the techniques of helioseismology.
In the 1960s it was discovered that the surface of the Sun rises and falls with a period of five minutes.
This was discovered using spectroscopy and the Doppler Effect.
If a sound-emitting object moves away from your ear, you hear the pitch of the sound drop; if it moves toward you, the pitch rises.
A similar effect occurs with light waves: a receding object appears redder (known as redshift) and an approaching one, bluer (known as blueshift).

At the end of the 1970s the Sun was observed from the Earth’s south pole during the Antarctic summer for a continuous 4.5 days, when scientists were able to measure its pulsation to a precision of 2cm per second.
Since then, international networks of telescopes and space-based instruments have monitored the movement of our nearest star.
The Sun’s oscillations are caused by sound waves trapped inside the solar interior, which are in turn produced by the convective turbulence in the outer 30% of the Sun.
We see the effects of this turbulence as granulation on the surface of the photosphere.
The sound waves caused by pressure fluctuations in this turbulence reflect inwards once they hit the Sun’s surface.
The inward-moving waves are refracted (their direction is bent) by the increase of the speed of sound – due to the rising temperature within the Sun – before eventually returning to the surface.
The combination of all these internal sound waves makes the Sun vibrate in millions of different ways.
It is at this point that the mathematics becomes complicated, but it is enough to say that helioseismologists can use these patterns to infer the internal structure of the Sun in similar ways that seismologists use earthquake patterns to understand Earth’s interior.
Stars

Helioseismology can be thought of as a branch of asteroseismology.
As the Sun is much closer than other stars, it has been easier to study how it vibrates.
And what helioseismologists have learned about the Sun’s interior can be transferred to other stars of different types, with a view to probing their interiors using sound waves.
The variability of some stars has been known for more than 400 years, since David Fabricus recorded the changing brightness of the most famous variable star, Mira, in 1596.
Mira still pulsates today, which means that its variability is being sustained by something – if it wasn’t, the bulk of the star itself would damp out the motions.

This sustaining mechanism can be thought of as a layer within the star, opaque to certain wavelengths of radiation, that is trying to escape into space.
This layer is forced to absorb the radiation, heating and expanding it, making the star swell up beyond its normal size.
As the opaque layer heats up it becomes ionized, which makes it less opaque and allows the radiation to get through.
As it is no longer absorbing radiation, the now transparent layer cools back down and is forced to contract by the weight of the gas above it, becoming opaque and starting the entire process once again.
Another class of vibrating stars was discovered by Donald Kurtz at the University of Central Lancashire some 25 years ago.
He was studying Przybylski’s star – an unusual, near-main sequence star whose spectrum revealed it to contain 100,000 times more metals than the Sun, the type of abundances you would expect from a much older and more evolved star.
Conventional wisdom dictated that such a star should not pulsate, as its very strong magnetic field would stabilise it.
Kurtz discovered that Przybylski’s star vibrated with a frequency of 12 minutes – energetic indeed for a star heavier than the Sun.
Since then, more than 30 of these ‘rapidly oscillating peculiar A stars’ have been discovered, with pulsation periods ranging from five to 20 minutes, most of them by Kurtz and his team.
They are amongst the strangest stars yet discovered by astronomers.
Asteroseismology is far from being a mature field of study, but advances in instrumentation are accelerating its evolution.
For Alpha Centauri A, for example (the nearest star visible to the naked eye), astronomers can now measure the speed of the surface oscillations to an accuracy of 2cm per second.
4 stars that make sounds
1
Xi Hydrae

This star is 160 lightyears away, and measures around 10 times the diameter of the Sun, with about 60 times its luminosity. It oscillates with several periods of around three hours, like a ‘sub ultra bass’ instrument. It is the most massive star in which solar-type oscillations have been discovered.
2
Alpha Centauri A

The nearest star to the Sun pulsates every seven minutes – very similar to the Sun. With a frequency of around 35cm per second, these pulsations make the star’s surface rise and fall by around 40m. This is an amazing observation on a star that measures some 1.75 million km across.
3
BPM 37093

BPM 37093 is a white dwarf – the core of a dead star – that is located 50 lightyears away. It pulses as its brightness changes by around one per cent every five to 10 minutes, allowing astronomers to deduce that this star has partially crystallised into a gigantic diamond.
4
Vela Pulsar

One of the youngest pulsars yet found, this supernova remnant rotates every 89 milliseconds. More than a dozen ‘glitches’ have been observed where it spins slightly faster by a matter of nanoseconds. This is caused by starquakes: huge stresses being released in a solid crust over a ‘superfluid’ interior.
Black holes
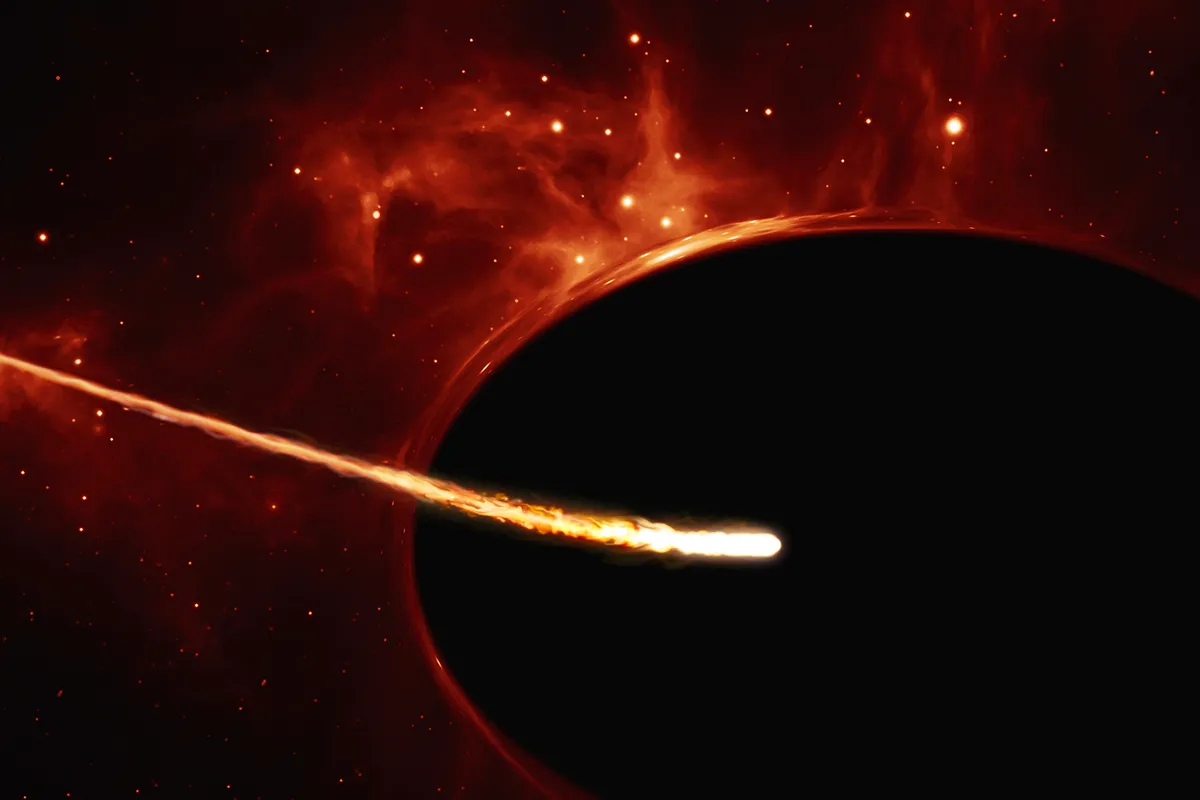
It is not just the stars that produce sounds; other, more exotic phenomena have recently been shown to be ‘noisy’.
In September 2003 a media fanfare accompanied the announcement of the discovery of sound waves emanating from a supermassive black hole in the Perseus cluster of galaxies about 250 million lightyears away.
The observations were made by NASA’s Earth-orbiting Chandra X-Ray Observatory, which collected 53 hours of data on the phenomenon.
Black holes themselves are invisible, but they create a riotous, chaotic and energetic environment in their immediate vicinity, as in-falling matter is accelerated to close to the speed of light.
All types of radiation have been detected from these regions, from radio waves right through the electromagnetic spectrum to high-energy X-rays.
Many black holes have also been discovered to emit powerful jets of matter along their axes of rotation.
Perseus cluster

In the Perseus cluster, these jets are thought to be slamming into the thin intergalactic gas surrounding the cluster.
The results of this ongoing collision were revealed by the observations from Chandra to be immense circular ripples, spreading out in all directions, like those created by an object dropped into water.
These ripples, or pressure waves, obey the same laws as sound waves and, even though no one can hear them, they are true sound waves travelling through very thin gas in space.
Just as on Earth, where the pitch of a musical note created in air depends on either the sound’s wavelength, or the distance between each ripple of compression, the same is true of the Perseus black hole.
The ripples seen by Chandra are 35,000 lightyears apart. Dubbed ‘the deepest note in the Universe’, this is what you would get if you could make a musical note 57 octaves below middle C.

The sounds made by black holes have not been directly heard – they have not travelled through space to the Earth as sound waves.
Rather we can see the effect they have on the gas surrounding them using X-rays, like using visible light to witness the waves when a stone is dropped into a pond.
A normal piano covers seven octaves of musical notes, but the Perseus black hole sings some 57 octaves below middle C – or more than a million billion times deeper than the range of human hearing.
These sound waves are more than a curiosity, and may help explain how galactic clusters grow.
It has been a long-standing puzzle to astronomers as to why there should be so much hot gas in clusters of galaxies.
It would normally be expected to cool and fall towards the centre of the galaxies, forming lots of new stars as it clumps together.

Heat from a large central black hole could be the answer. The jets of material streaming from the Perseus black hole form cavities in the thin cluster gas as they collide with it.
Sound waves then travel outwards as huge ripples.
The sheer amount of energy required to create these cavities is equivalent to around 100 million supernovae, a lot of which is carried away in sound waves.
These then warm the cluster gas as they dissipate – preventing it from cooling and forming stars.
The Big Bang
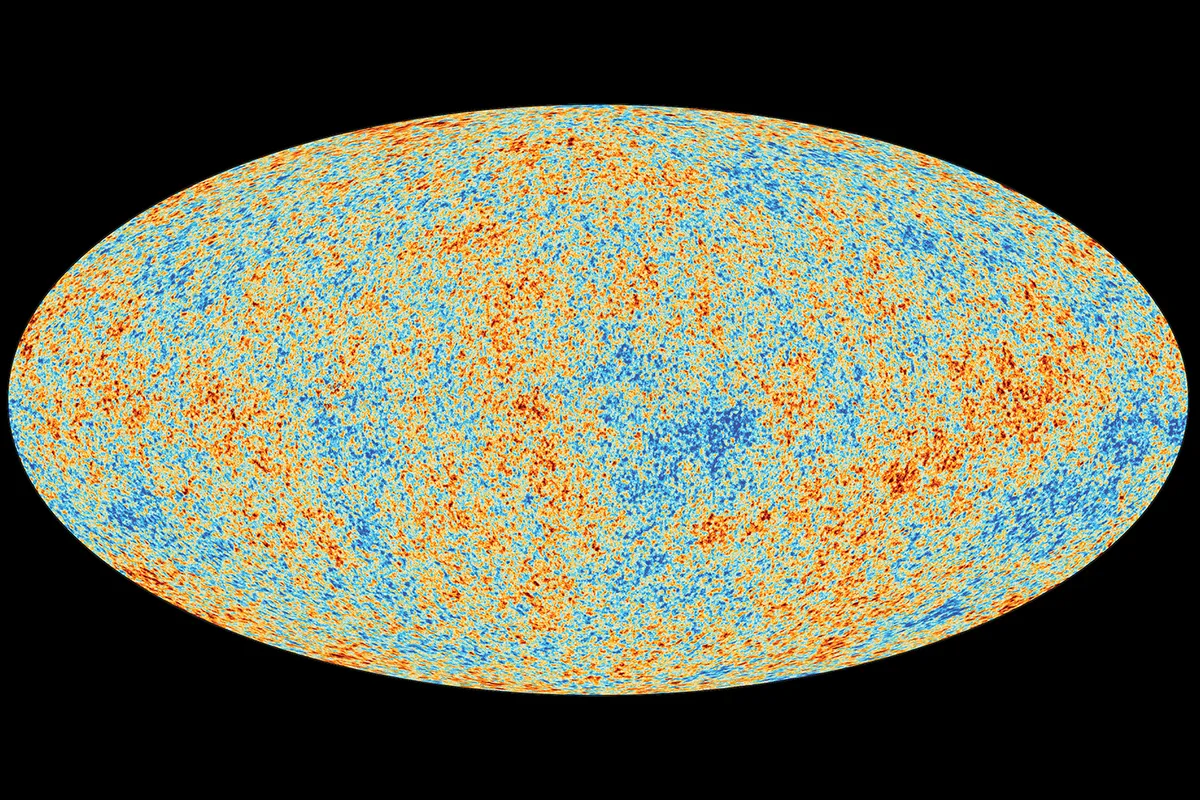
Precise measurements of the Cosmic Microwave Background give a view of the Universe around 400,000 years after the Big Bang, just as it had cooled enough to allow the first atoms to form from the primordial plasma.
Detailed studies of the minute variations in this background radiation suggest that, at this stage of the early Universe, titanic acoustic waves propagated through this plasma.
Stars and galaxies had yet to form and all matter existed as a kind of hot fog in the rapidly expanding Universe.
It is this foggy, thin gas that allowed sound waves to form and propagate.
Tiny differences in the data from the Wilkinson Microwave Anisotropy Probe (WMAP) show peaks and troughs in these sound waves.
So did the Big Bang actually bang? Analysis of the WMAP data by US astronomer Mark Whittle suggests that the Big Bang was initially silent, but the noise quickly grew into a "descending scream", followed by a "deep roar" and ending in a "deafening hiss", with a peak volume of around 110 decibels – equivalent to the noise of a rock concert.
These sound waves had wavelengths of around 20,000 lightyears, around 50 octaves below the range of human hearing.
Their discovery and measurement marks the beginning of a new era in precision cosmology.