Have you ever wondered how stars shine?
It's awe-inspiring to stand under a clear night sky and realise that every one of the countless pin-points of light is a blazing star, many times the size of the whole world.
Some stars are as old as the Universe itself and, like our own Sun, each star creates enough light to be seen across thousands of lightyears.
You may already be aware what light is: a self-perpetuating disturbance in the electric and magnetic fields that pervade all space.
How does what's happening inside a star end up disturbing those fields, creating the light that eventually disturbs the cells in our retinas?
Take a step back and read our guide explaining What is light?

Stars don't burn
On a really hot summer’s day, the Sun seems to burn like a furnace, which is quite impressive considering it is 93 million miles away.
But ‘burn’ is the wrong word here.
Lord Kelvin, one of the founding fathers of thermal physics, realised that burning – that is, a chemical reaction involving oxygen – couldn’t sustain the Sun’s huge power output.
His suggestion, the only feasible mechanism known to Victorian physicists, was that the Sun generated heat by gradually collapsing under its own gravity.

This image was captured by NASA's Solar and Heliospheric Observatory on 4 January 2002. Credit: NASA/SOHO
A bicycle pump gets hot when you use it because gas heats up when it is squeezed.
Kelvin worked out that gravity squeezing the Sun could keep it hot for about 20 million years.
But geologists at the time argued, correctly, that the Solar System was much older.
So there had to be some other, as-yet-undiscovered source of the Sun’s power.
Brilliant astrophysicist Arthur Eddington solved the enigma in the early 20th century, proposing that nuclear rather than chemical reactions could power the Sun for billions of years.
Details of those nuclear reactions were finally worked out by Hans Bethe in the 1930s, for which he received the 1967 Nobel prize
But just how do nuclear reactions create light that makes stars shine?
Every charged object (such as a balloon that has been rubbed on nylon to give it ‘static electricity’, which is also called charge) is surrounded by an electric field that stretches out endlessly in all directions.

If you shake that charged object, you create ripples in its surrounding field and, as James Clerk Maxwell realised, a disturbance in an electric and/or magnetic field is called light, though some frequencies of disturbance are given other names, such as ‘radio waves’ or ‘X-rays’.
If you could shake an electric charge up and down about 500 million million times per second, you would create yellow light.
Charged particles do get shaken at that sort of rate during processes that take place inside atoms and inside stars.
All starlight comes from electric charges being shaken, in various ways.
What are stars made of?

We should begin by considering what stars are made of, since that’s the stuff responsible for generating light.
Ordinary matter, such as iron or helium or economy-range lasagne, is made of atoms.
All atoms contain charged particles: a positively charged nucleus surrounded by a cloud of negatively charged electrons (the grey cloud in the picture of a helium atom shown on the left).
These equal and opposite charges attract, so the electron cloud tends to stay near the nucleus, though they can be separated quite easily.
The nucleus is itself composed of smaller parts: positively charged particles called protons (shown in red in the image above), stuck together by sticky neutrons (shown in blue) which, as their name suggests, are neutral (not charged).
With this knowledge, we can investigate the processes inside stars that conspire to shake the charged particles.
The mechanism that makes ordinary main-sequence stars shine is the sticking together of hydrogen nuclei to make helium nuclei, a process called nuclear fusion – see how other stars do it in ‘How other types of stars shine’, below.
The nucleus of a hydrogen atom is comprised of just one proton.
So ‘hydrogen nucleus’ is another way to say ‘proton’.
A helium nucleus, the end product of the process we’re going to examine, is a ball of four particles: two protons and two neutrons.
To manufacture a helium nucleus out of hydrogen, four protons must get collected together and two of them must metamorphose into neutrons.
These things happen in several different stages, some of which generate light.

Where particles come in
Let’s skip the very first stage, where two protons happen to collide and the impact makes one of them turn into a neutron by squirting out its positive charge in the form of a tiny particle called a positron.
The resulting combined object – a proton stuck to a neutron – is called a deuteron.
So we take up the story where a deuteron has formed somewhere in the heart of a star, surrounded by octillions of tonnes of protons zooming about like fairy cakes at a chimps’ tea party.
Sooner or later one of those protons is going to collide head-on with our deuteron, bringing it close enough to feel the mysterious sticky attraction of the neutron.
The neutron’s stickiness is called the ‘strong nuclear force’.
It is one of the four fundamental forces of nature, the others being electromagnetism, the weak nuclear force and gravity.
Like gravity, the strong nuclear force pulls things together, but it operates over much shorter distances.

By analogy, rather than thinking about a proton being pulled by the nuclear force of a neutron, let’s imagine a spacecraft, like the Cassini mission to Saturn, being pulled by the gravity of a planet.
On the way to Saturn, Cassini passed Jupiter.
While approaching the gas giant, Cassini was falling, allowing the planet’s gravity to accelerate it.
But it didn’t get trapped on Jupiter like a proton stuck to a neutron.
As it fell, it sped up, and the extra speed was enough to take it on past Jupiter, away from its gravitational influence.
Next, Cassini approached Saturn, and fell once more.
Again, the craft gained too much speed to stay in orbit so, to avoid over-shooting this planet too, it had to lose some of the kinetic energy of its motion.
In principle, the mission’s designers had a choice of two possible methods for achieving this: fling out some fast-moving fuel from its retrorockets, letting the ejected fuel carry away the excess of energy and leaving Cassini in orbit around Saturn, or convert its kinetic energy into heat by crashing.
Either method would have left Cassini gravitationally bound to the planet. True to their legendary intelligence, the rocket scientists chose the first option.
What can we learn from Cassini?
That an attraction isn’t enough to bind two free bodies together; they must also jettison some energy to avoid flying apart again.
So, if a proton falls towards a deuteron and they end up stuck together, we can infer that they must have jettisoned some energy.
In this case, it’s not rocket fuel that they cast out, but light.
The resulting body, composed of two protons and a neutron, is called a helium-3 nucleus.
After some more collisions, it will eventually acquire another neutron to form an ordinary helium nucleus, releasing more light energy in the process.

Quantum weirdness
By thinking about the energy possessed by particles before and after they stick together, we have been able to deduce that energy must be released during nuclear fusion.
But this doesn’t explain how the process creates the light.
The explanation must involve shaking electric charges.
Protons are charged particles, but why do they shake during nuclear fusion? The answer is very strange indeed.
When examined in fine detail, the laws of nature are weirder than they appear on a large scale.
The eccentric rules of quantum physics allow a microscopic object to exist in many different places at once, like the two tiny electrons in the helium atom depicted further up this page, which are smeared out into a grey cloud.
Erwin Schrödinger, a pioneer of quantum physics, demonstrated its bizarreness by telling the story of a cat, left in a box that contains some lethal quantum-mechanical device that may or may not activate.
The cat may or may not be killed. Quantum laws insist that, while it remains unobserved, the cat exists in a mixed state, simultaneously alive and dead.

It is the same for the proton during nuclear fusion. It could end up bound to the deuteron, or it might just zoom on past.
For a short while, its fate is uncertain, and it exists in a mixture of the two conditions: bound and free.
These two states of the proton – bound and free – have different amounts of energy.
The laws of quantum physics dictate that, when an object is in a mixture of two states with different energies, it oscillates back and forth, one moment appearing more like the first state, the next more like the second.
This is the mechanism that shakes the proton, making it shake the electric field, creating light.

Whole lotta shakin’
If this was the end of the story, main-sequence stars would shine with ‘gamma rays’, the ultra-high-frequency colour of light produced by nuclear fusion.
In fact, buried deep in the stellar interior, the gamma radiation generally doesn’t escape the star.
Instead, its oscillating electric and magnetic fields shake a nearby charged particle (perhaps another proton or an electron) giving said particle some energy, which it quickly loses again by creating more light of a different colour that matches the frequency of its motion – for more detail on this see ‘Studying starlight’, below.
So stars shine because they're a crowd of charged particles that shake electromagnetic fields which, in turn, buffet other charged particles.
Eventually, electromagnetic ripples reach the surface and flee, unimpeded by any charges in the near-emptiness of space.
How other types of stars shine
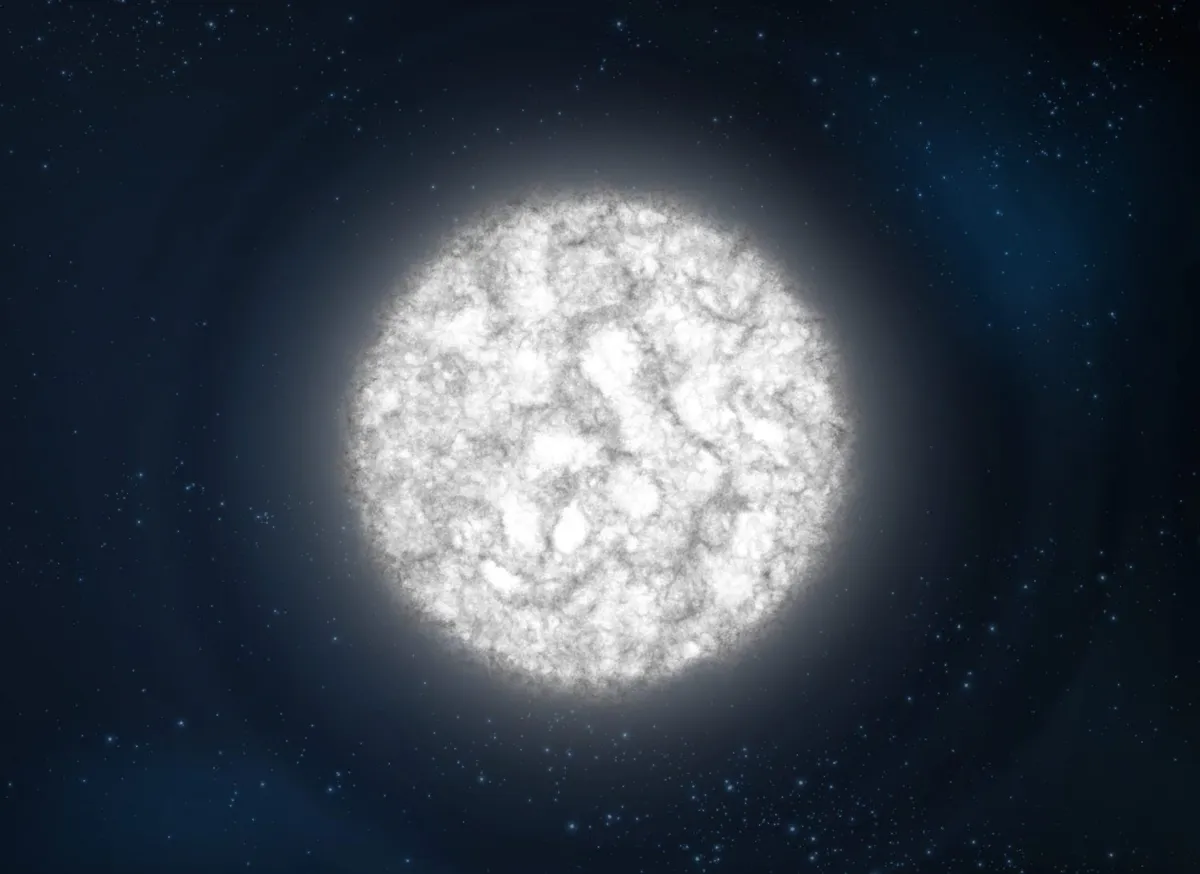
7activestudio / Getty Images
Within a star, charged particles shove each other about like ice hockey players in an averagely friendly game, shaking the electric field that accompanies them, creating light.
The kinetic energy of all this subatomic jiggling is what we call heat.
The source of that energy, in main-sequence stars, is nuclear fusion. But some other celestial bodies are heated in different ways.
White dwarfs and neutron stars have no energy source.
Their nuclei fused long ago, during their earlier careers as ordinary stars.
Now they are losing energy, as their charged particles continue to make ripples in the electromagnetic fields and gradually slow down as a result.
But these stars have enough kinetic energy (heat) stored up to keep them shining for countless billions of years.
Black holes have a unique way of generating heat. Any gas, dust or star that approaches a black hole falls fast.
On encountering other material, in the swirling mass of doomed matter known as the black hole’s accretion disc, they smash together with great violence, vigorously shaking the electric fields of their constituent charged particles.
This is why, paradoxically, black holes do give off light.

On the large scale, we observe all this violence as the extreme heating of the accretion disc, which shines brightly with X-ray light as a result.
Then there are brown dwarfs, a largely unnoticed class of stars named in honour of their underwhelming glimmer of light, mostly infrared in colour.
The heat responsible for their meagre luminance stems from the gradual compression of their gas, as they slowly shrink, due to gravity.
This is exactly the process of stellar heating proposed by Lord Kelvin in the pre-nuclear Victorian era. So he wasn’t entirely wrong after all.
Studying starlight

Encoded in starlight is information about the motion of the particles that created it.
Because the colour of light produced by a charged particle matches the frequency at which it was shaken, astronomers can study the motion of a star’s constituent particles using ‘spectroscopy’: measuring the brightness of each colour in the mixture of light.
Almost every astronomical research project uses spectroscopy.
A star’s overall average colour tells its temperature because it reveals the average rate of motion of its particles.
This is why some stars are red and some stars are blue.

temperatures around 3,000°C and
the hottest are white at a toasty 40,000°C. Credit: Christian Darkin / Science
Photo Library
Although our models of stars are fairly complete, accurately reproducing the observed stellar temperatures, some peculiar stars such as ‘blue stragglers’ need more thought, being hotter than predicted by the basic model of stellar evolution.
Observations of the colours of starlight in globular cluster M10 support the idea that blue stragglers form when so-called ‘main-sequence’ stars either collide or steal gas from a binary partner.
When negatively-charged electrons are trapped within an atom, as they are in the cooler outer regions of a star’s atmosphere, they are limited to possess only a small number of possible energies, so that they can only emit or absorb a certain set of well-defined colours of light.
Recognising these discrete sets of colours in starlight, known as ‘line spectra’, allows us to identify which atoms are present.
For instance, one study of line spectra shows that iron is more abundant in stars closer to the centre of the Milky Way, suggesting that stellar evolution and the production of chemical elements occurs differently in different parts of a galaxy.
This article appeared in the August 2013 issue of BBC Sky at Night Magazine.